Multimodal sensing conductive organohydrogel electronics based on chitosan-encapsulated MXene nanocomposites for deep learning-enhanced ball sports recognition
Abstract
Conductive hydrogels have drawn significant attention as smart sensing systems for flexible electronics. However, challenges remain in fabricating multimodal electronics that simultaneously achieve ultrastretchability, conformal adhesion, environmental adaptability, self-healing, and high-performance sensing for electrophysiological signal detection. In this study, a nanocomposite organohydrogel with these features is developed by incorporating chitosan-encapsulated MXene nanosheets into a polyacrylamide network within a phytic acid (PA)/glycerol (GL)/water trisolvent system, aiming to create a multimodal sensing platform. The synergy between hydrogen bonds and electrostatic interactions endows the organohydrogel with exceptional properties, including ultrastretchability (2,800%), robust adhesion (70.6 kPa on paper), and self-healing ability. The combination of PA and GL not only enhances the organohydrogel’s environmental adaptability (-30 to 60 °C) to meet diverse application requirements but also improves its conductivity. These remarkable features enable the organohydrogel to function as a multimodal sensor capable of detecting multiple stimuli (strain and temperature) with high sensitivity and strong robustness against external disturbances. Moreover, it serves as a reliable electrode for electromyography signal detection, providing a high signal-to-noise ratio and low interfacial impedance. By integrating deep learning algorithms, the organohydrogel sensing system achieves 100% accuracy in ball sports identification, showcasing its potential for multimodal sensing platforms.
Keywords
INTRODUCTION
Flexible electronic platforms that replicate the features and functions of natural skin by converting external stimuli (e.g., strain and temperature) into electrical signals have garnered significant attention and find broad applications in electronic skin, artificial intelligence, and healthcare monitoring[1-4]. Among various options, conductive hydrogels (CHs) are particularly promising due to their tissue-like properties, stimuli responsiveness, and satisfactory conductivity, making them an ideal platform for flexible electronics[5]. However, practical applications often involve exposure to extreme thermal environments (e.g., both cold and hot conditions), which pose significant challenges to the mechanical properties and functionality of CH-based electronics with high water content, severely limiting their operational range and long-term applicability[6]. Therefore, developing CH-based electronics with enhanced environmental adaptability, while maintaining inherent advantages of hydrogels, is critical for expanding their practical applications.
Typically, to improve the water retention ability, introducing non-volatile solvents [i.e., glycerol (GL), ethylene glycol, or dimethyl sulfoxide] with low vapor pressure into the hydrogel system is one of the efficient ways to lock water molecules within the polymeric network[7]. For example, an organohydrogel made from a GL/water mixture maintained flexibility and excellent sensory behavior between -18 and
Biomass polysaccharides, such as cellulose[10] and chitosan (CS)[15], contain abundant hydroxyl and carboxyl groups, enabling MXene nanosheets to be evenly integrated into the hydrogel through hydrogen bonds. The successful stabilization of MXene nanosheets driven by cellulose nanocrystals to construct stable CHs illustrates this approach[16]. Therefore, incorporating polysaccharides into the hydrogel can ensure the stable dispersion of MXene nanosheets, which is advantageous to the construction of perfect conductive path. Moreover, while recent studies on MXene-based hydrogels primarily focus on improving mechanical or electrical properties, they often overlook other essential properties such as self-adhesion and self-healing, which are crucial for the practical use of CHs. Therefore, constructing stable MXene-composited organohydrogel with multifunctionality via a simple approach remains a significant challenge.
Phytic acid (PA) is a natural organic acid containing six phosphate groups, which can be extracted from plant seeds and possesses excellent biocompatibility. Generally, dynamic hydrogen bonds between PA and matrix play a significant role in the properties of the organohydrogel, endowing it with excellent self-healing ability[17]. Moreover, PA has abundant donor and acceptor sites of hydrogen bonds, which improves the possibility of PA to bind with water molecules via hydrogen bonds, inhibiting the crystallization and evaporation of water in the organohydrogel[18]. Furthermore, PA is an electrolyte and abundant free H+ can be released after being dissolved in water, improving the conductivity of organohydrogel[19]. Hence, it is anticipated that incorporating PA into the organohydrogel would synchronously produce high conductivity and environmental stability to facilitate the development of flexible sensors.
With the rapid development of technologies such as big data, there is an increasing demand for high-performance electronics to enhance data acquisition and information processing efficiency, particularly in the field of human detection[20]. To capture complex human activities, multiple flexible sensors need to be integrated into different parts of the body[21]. However, individual differences pose significant challenges to the reliability and multifunctionality of such sensing systems. Recently, advanced deep learning algorithms (DLAs) have been introduced into the realm of flexible sensors, which employ the collected high-quality data to build models, demonstrating the correlations and faint discrepancies among different inputs and improving the accuracy of decision-making[22]. By leveraging deep learning, wearable sensing systems are expected to provide more accurate predictions and enhance sports recognition accuracy. Therefore, integrating advanced DLAs into wearable MXene-composited organohydrogels represents a promising approach for reliable sports recognition.
Herein, we introduce a new approach for improving nanocomposite organohydrogel functionality through leveraging CS to protect and stabilize dispersed MXene nanosheets. The MXene nanosheets are effectively encapsulated by CS moleculars through hydrogen bonds and electrostatic interactions, forming a passivation layer that enhances the dispersibility and stability of MXene and facilitating the polymerization of acrylamide (AM) monomers in a PA/GL/water trisolvent system. The MXene-nanocomposited organohydrogel exhibits ultrastretchability, environmental stability, reversible adhesiveness, self-healing ability, and reliable dual-sensing capabilities for strain and temperature. The formation of homogeneous organohydrogels and their resulting ultrastretchability (2,800%), satisfactory adhesion strength, and self-healing ability are attributed to dynamic reversible interactions between PA, CS, and the negatively charged MXene nanosheets. The PA/GL/H2O trisolvent system ensures the organohydrogel’s environmental stability across extreme temperatures (-30 to 60 °C). The combination of PA and MXene nanosheets endows the organohydrogel with favorable conductivity, reliable thermosensation, and the ability to respond to different deformations, enabling dual-sensing of strain and temperature. Remarkably, these multifunctional sensors can detect complex human activities under harsh temperatures and subtle electromyogram signals. Moreover, advanced DLAs are integrated with the organohydrogel sensors, enabling an intelligent sports recognition platform with 100% accuracy in identifying different ball sports.
EXPERIMENTAL
Materials
AM, GL, ammonium persulfate (APS), N, N′-methylene-bis-acrylamide (MBA), lithium fluoride (LiF), and PA (50% in H2O) were purchased from Aladdin (Shanghai, China). CS (degree of deacetylation ≥ 95%, and 100-200 mPa·s) was provided by Macklin Biochemical CO., Ltd. (Shanghai, China). MAX phase (Ti3AlC2, 400 mesh) was bought from 11 Technology Co., Ltd (Jilin, China). Hydrochloric acid (HCl, 37 wt%) was acquired from Haohua Chemical Reagent Co., Ltd. (Luoyang, China).
Preparation of conductive PAM/CS/MXene nanocomposite organohydrogels
Multifunctional PAM/CS/MXene nanocomposite organohydrogels were prepared via free radical polymerization. Briefly, a certain amount of PA, GL and H2O was poured into a blue glass bottle and stirred in a 90 °C water bath for 30 min. Then, CS (0.2 g) was dispersed into the PA/GL/H2O dispersion (20 g) with constant magnetic stirring at ambient temperature for 2 h to obtain a uniform and stable dispersion. After cooling to the room temperature, AM (5 g) was added into the dispersion and stirred for 2 h until AM was completely dissolved. Subsequently, different contents of MXene (the preparation process is detailed in the Supplementary Materials) and MBA solution (0.325 mL, 1 wt%) were sequentially employed into the abovementioned dispersion and magnetically stirred for 2 h. The MXene content was set as 0, 0.1, 0.2, and 0.3 mg·g-1. After degassing treatment, APS solution (0.08 mL, 10 wt%) was added into the mixture and uniformly stirred for 30 s. Then, the precursor solution was promptly poured into self-made molds and put at 60 °C for 1 h to achieve the PAM/CS/MXene nanocomposite organohydrogels, which were defined as PCM organohydrogel. The CS content was changed from 0 to 0.015 g·g-1. For convenience, the prepared nanocomposite organohydrogel was labeled as PCMx, where x represented the weight ratio of MXene to total solvent (mg·g-1). As a reference, PCM hydrogel was fabricated via the same method.
RESULTS AND DISCUSSION
The ingenious design strategy and fabrication procedure of PCM nanocomposite organohydrogels are depicted in Figure 1A, which is prepared via the one-pot polymerization by incorporating CS-encapsulated MXene nanosheets into a PAM organohydrogel within a PA/GL/water trisolvent system. The MXene nanosheets were produced through etching and exfoliating Ti3AlC2 with a LiF/HCl solution, resulting in high-quality, transparent nanosheets with a lateral size of 300 nm and an average thickness of 3 nm, can be achieved [Figure 1B and C]. X-ray diffraction (XRD) patterns confirmed the successful synthesis of MXene nanosheets [Supplementary Figure 1]. PA plays a dual role in the organohydrogel: providing an acidic environment to ensure the complete dissolution of CS and acting as a source of free mobile ions to enhance conductivity. Additionally, PA can anchor and crosslink with CS chains through electrostatic interaction and hydrogen bonds[18]. Particularly, the tendency of nanofiller-type conductive phases to slip and recombine during deformation is a key challenge in flexible matrices[23]. Here, abundant -NH2 and -OH functional groups of CS can form robust noncovalent interactions (hydrogen bonds and electrostatic interactions) with the functional groups (-OH, -F, -O, etc.) on MXene nanosheets, making CS an ideal candidate for creating stable conductive MXene nanocomplexes. To validate the protective role of CS in stabilizing MXene nanosheets in aqueous environments, both CS-MXene and bare MXene colloidal dispersions were stored for 10 days at ambient temperature [Supplementary Figure 2]. This enhancement in stability improves the homogeneous distribution of MXene nanosheets within the organohydrogel, laying the groundwork for continuous and stable conductive pathways. Meanwhile, the incorporation of non-volatile GL can further enhance the environmental stability of the hydrogel.
Figure 1. (A) Design and preparation process of the PCM organohydrogel; (B) TEM and (C) AFM images of MXene; (D) SEM image and (E) elemental distribution of the PCM hydrogel; (F) ESP distribution of PA, PAM, CS, and PAM-CS-PA-GL-H2O; (G) DFT optimized structures and Eint calculations of different components in the PCM organohydrogel. PCM: PAM/CS/MXene; TEM: transmission electron microscopy; AFM: atomic force microscopy; SEM: scanning electron microscopy; ESP: electrostatic potential; PA: phytic acid; PAM: polyacrylamide; CS: chitosan; GL: glycerol; DFT: density functional theory.
Scanning electron microscopy (SEM) images of freeze-dried PCM hydrogels [Figure 1D] present the typical interconnected microporous architecture, indicating good dispersion of MXene nanosheets within the hydrogel matrix rather than aggregation [Supplementary Figure 3], which is beneficial to excellent stretchability and improved sensing response[13]. Due to the small size of the MXene nanosheets, they are challenging to observe directly. However, energy dispersive spectroscopy (EDS) mapping images confirm the presence and uniform distribution of MXene nanosheets within the PCM hydrogel, as evidenced by the detection of C, N, O, and Ti elements [Figure 1E]. The chemical structure of PCM organohydrogel is analyzed using Fourier transform infrared (FTIR) spectroscopy [Supplementary Figure 4]. To further delve into the mechanism of interactions between different components in the PCM organohydrogel, density functional theory (DFT) calculations were performed to simulate the molecular structure, determine the interaction energy (Eint), and verify the proposed interactions. The electrostatic potential (ESP) distribution reveals that the N+ of CS interacts strongly with amide group of PAM and -P(OH)3 of PA [Figure 1G]. The
PCM organohydrogels demonstrate high stretchability, withstanding direct and twisted stretching up to 2,800% and 2,200% strain without fracture, respectively [Figure 2A]. Furthermore, the PCM organohydrogel can support a 100 g weight without significant damage [Figure 2B], showcasing its excellent toughness. Mechanical properties of PCM organohydrogels were further analyzed as a function of MXene content [Figure 2C], and the incorporation of MXene enhances both tensile strength and elongation at break, with these properties peaking at an optimized MXene content and declining at higher concentrations [Figure 2D]. Here, the MXene content dependent mechanical properties can be explained as follows: when the MXene content is relatively low (< 0.2 mg·g-1), secondary crosslinking occurs between the MXene nanosheets and the organohydrogel framework through the abundant hydrophilic functional groups of MXene. This promotes the uniform dispersion of MXene in the hydrogel system and increases crosslinking density, thereby improving tensile strength and elongation at break[24]. However, when the MXene content rises to 0.3 mg·g-1, excess MXene nanosheets tend to aggregate, disrupting the uniformity of the hydrogel and reducing tensile strength and elongation at break[25]. As a result, the PCM organohydrogel with the optimized MXene content of 0.2 mg·g-1 exhibits a tensile strength of 80 kPa and an elongation at break of 2,800%, indicating that the incorporation of CS can improve mechanical properties of the nanocomposite organohydrogel [Supplementary Figure 8]. Moreover, incorporating MXene nanosheets also enhances conductivity of the PCM organohydrogel due to the excellent metallic conductivity of MXene and its ability to provide sufficient electron transport channels in the organohydrogel [Supplementary Figure 9]. Based on the optimal balance between mechanical properties and conductivity, the PCM0.2 organohydrogel is selected for further experiments.
Figure 2. Mechanical properties of PCM organohydrogels. Images exhibiting that the PCM organohydrogel can (A) withstand large direct stretching and twisted stretching and (B) lift a 100 g weight; (C) The tensile stress-strain curves and (D) corresponding elongation at break and tensile strength of PCM organohydrogels with different MXene contents; (E) Continuous loading-unloading curves of the PCM organohydrogel under increased strains without rest time and (F) the corresponding Uhys; (G) Twenty successive loading-unloading curves of the PCM organohydrogel with a preset strain of 500% and (H) the corresponding Uhys. PCM: PAM/CS/MXene.
Cyclic loading-unloading tests were conducted to further investigate the energy dissipation behavior and anti-fatigue ability of PCM organohydrogels. Figure 2E exhibits consecutive loading-unloading measurements of the PCM organohydrogel under increased strains, where clear hysteresis loops are observed during the loading-unloading cycles. As the tensile strain increases from 100% to 1,000%, the dissipated energy (Uhys) of the PCM organohydrogel rises from 2.7 to 37.2 kJ·m-3 [Figure 2F]. Meanwhile, the subsequent loading curve does not completely overlap with the previous unloading curve, which is attributed to the partial quick re-association of dissociated noncovalent bond interactions during loading, contributing to the energy dissipation[26]. Exceptional fatigue resistance is critical for ensuring the cycle stability and prolonged service life of CH-based sensors. To evaluate the anti-fatigue ability, the PCM organohydrogel was subjected to 20 consecutive loading-unloading cycles. The PCM organohydrogel presents a pronounced hysteresis loop with the Uhys of 46.8 kJ·m-3 in the first cycle [Figure 2G], which is attributed to the rearrangement of polymer chains and multiple noncovalent bond interactions within the PCM organohydrogel[27]. However, hysteresis loops of the subsequent nineteen cycles overlap almost perfectly, and the Uhys stabilizes at approximately 5.2 kJ·m-3 [Figure 2H]. This stable energy dissipation across cycles highlights the excellent fatigue resistance of the PCM organohydrogel, which is attributed to its rapid self-recovery behavior[28]. This ability ensures the hydrogel’s durability and effectiveness as a flexible, long-lasting strain sensor for wearable electronics.
Reliable self-adhesion behaviors of the PCM organohydrogels are crucial for their practical applications as wearable strain sensors, which can effectively enhance the interfacial connection and ensure accurate stable signal collection[9]. As depicted in Figure 3A, the PCM organohydrogel can readily adhere to a wide range of substrates, including wood, iron, rubber, pigskin, plastic, and paper. Meanwhile, it presents excellent conformality to fit into curved contour surface, such as human skin, making it ideal for detecting complex movements [Figure 3B]. Impressively, the PCM organohydrogel can be easily peeled off without leaving residue or causing irritation to the skin after 20 peeling cycles. The adhesion strength of the PCM organohydrogel was quantitatively evaluated through lap shear measurements [Figure 3C]. As seen in Supplementary Figure 10, the adhesion strength is closely correlated with the CS content in the PCM organohydrogel. The improvement in adhesiveness is ascribed to the introduction of CS, which enhances cohesion within the gel system[29]. The universal adhesiveness of the PCM organohydrogel is demonstrated on a variety of substrates, highlighting its versatility and effectiveness. The PCM organohydrogel exhibits strong adhesion to paper, wood, iron, glass, and pigskin, and the maximum adhesion strengths reach 70.6, 38.9, 32.4, 24.7, and 16.7 kPa, respectively [Figure 3D]. This broad range of adhesive abilities suggests that the PCM organohydrogel can be applied in various practical settings. Furthermore, the repeatable and reproducible adhesion abilities of the PCM organohydrogel to various substrates are demonstrated through multiple cycles. There is no noticeable decrease in adhesion strength even after four cycles [Figure 3E], indicating that the PCM organohydrogel possesses excellent adhesion reusability. This remarkable reusability is attributed to the reversible interfacial interactions between the PCM organohydrogel and substrate surfaces, allowing the adhesion to be maintained even after repeated cycles [Supplementary Figure 11]. This feature will undoubtedly ensure the long-term effectiveness and durability of PCM organohydrogels in practical applications.
Figure 3. Self-adhesion and self-healing behaviors of the PCM organohydrogel. (A) Images demonstrating the tight adhesion of PCM organohydrogels to various materials; (B) Photographs exhibiting the excellent conformal adhesiveness of the PCM organohydrogel with no residue and no irritation on the human skin after 20 peeling cycles; (C) Schematic diagram of the lap shear measurements; (D) Adhesion strength of the PCM organohydrogel to different substrates; (E) Adhesion strength and reliability of the PCM organohydrogel to different substrates in four cycles; (F) Illustration of the self-healing feature of the PCM organohydrogel for a designed circuit; (G) Optical and (H) ultra-deep-field 3D images demonstrating the self-healing process of the PCM organohydrogel; (I) Tensile stress-strain curves and (J) the calculated HEs of the PCM organohydrogel with different healing time. PCM: PAM/CS/MXene; HEs: healing efficiencies.
The self-healing ability of PCM organohydrogels is demonstrated through macroscopic, microcosmic and tensile tests. As shown in Figure 3F, the lighted light-emitting diode (LED) in a simple circuit extinguishes synchronously once the organohydrogel is cut into two pieces, and then the LED is lit again immediately and the brightness can return to the original state after healing, giving an intuitive validation of the rapid self-healing ability. The self-healing process of PCM organohydrogels at the damaged surface is traced via an optical microscope [Figure 3G]. Two separate pieces of the PCM organohydrogel are recombined without the application of external stress. After healing for 24 h, the incision in the PCM organohydrogel nearly disappears [Figure 3H], demonstrating excellent self-healing behavior. Mechanical properties of the healed PCM organohydrogel were measured to further evaluate the self-healing effect, and the corresponding healing efficiency (HE) values were quantitatively calculated. As expected, the HE gradually increases with the healing time [Figure 3I] and reaches 60% for a healing time of 72 h [Figure 3J]. Impressively, the healed organohydrogel shows good fatigue resistance [Supplementary Figure 12], further confirming excellent self-healing ability and mechanical stability of the PCM organohydrogel. This outstanding self-healing ability and stable mechanical performance may stem from multiple reversible noncovalent bonds, such as hydrogen bonds between PAM chains, PA and PAM, PA and CS, PA and MXene, PAM and MXene as well as CS and MXene, and electrostatic interactions between CS and MXene nanosheets together with CS and PA within the organohydrogel system [Supplementary Figure 13]. This remarkable recovery ability underscores the potential of PCM organohydrogels for applications where durability and long-term functionality are critical, such as in flexible electronics.
Excellent environmental stability is vital for the practical application of organohydrogel, particularly when used as sensors in both extreme and mild environments. The incorporation of GL and PA in the PCM organohydrogel enables the formation of robust hydrogen bonds with water molecules within the network, significantly enhancing the environmental stability of the hydrogel and extending its potential applications. PCM organohydrogels are capable of sustaining twisting and large stretching deformations, and can even light up an LED indicator at extreme temperatures of -30 and 60 °C, as well as 25 °C [Figure 4A], visually demonstrating the excellent environmental stability. Furthermore, the favorable adhesion properties of PCM organohydrogels can also be well maintained over a wide temperature range from -30 to 60 °C [Figure 4B]. Temperature resilience of the PCM organohydrogel was probed through differential scanning calorimetry analyses [Supplementary Figure 14]. The outstanding environmental stability is attributed to the formation of strong hydrogen bonds between GL and water molecules, effectively reducing the saturated vapor pressure of water[30]. The hydrogen bonding network of adjacent molecules was explored through Raman spectroscopy. The bands between 3,000 to 3,800 cm-1 correspond to the O-H stretching vibration of water molecules and two type of water present in the spectra can be precisely distinguished[31], with the peaks located at about 3,450 and 3,200 cm-1 corresponding to strong and weak hydrogen bonded water molecules, respectively [Figure 4C]. Notably, when comparing the PCM hydrogel with the PCM organohydrogel, a shift of the characteristic peak from 3,345 to 3,366 cm-1 (blue shift) can be observed [Figure 4D]. This indicates that the strong hydrogen bonds between water molecules in the PCM organohydrogel are affected by the PA/GL/water trisolvent system. The ratio of strong to weak hydrogen bonds (Istrong/Iweak) decreases in the PCM organohydrogel [Supplementary Figure 15], suggesting a weakening of hydrogen bonds in the organohydrogel. The long-term stability of PCM organohydrogels is further assessed [Supplementary Figure 16].
Figure 4. (A) Images demonstrating that the PCM organohydrogel possesses excellent mechanical deformations and can light up a LED bulb at different environmental temperatures; (B) Photographs illustrating the excellent adhesion to wood and iron at different environmental temperatures; Raman spectra of (C) fitted O-H stretching vibration of water molecules with strong and weak-H bonds and (D) water molecules in the PCM hydrogel and PCM organohydrogel; (E) Fitted curve showing the ΔR/R0 value of the PCM organohydrogel as a function of temperature ranging from -30 to 60 °C; (F) Comparison of the PCM temperature sensor with other reported flexible temperature sensors in terms of temperature sensing range and TCR values. Stable and reproducible electrical sensing signals of the PCM organohydrogel through changing the temperature from 25 °C to (G) -10, -20, and -30 °C together with (H) 40, 50, and 60 °C; (I) Dynamic responses of the PCM organohydrogel under constant temperature induced by a hair dryer’s heat flow. PCM: PAM/CS/MXene; LED: light-emitting diode; TCR: temperature coefficient of resistance.
High thermal effect of MXene and PA enables PCM organohydrogels to function as effective temperature sensors for detecting thermal stimuli [Supplementary Figure 17]. The sensitivity of the thermal response is quantified by the temperature coefficient of resistance (TCR). Relative resistance variation (ΔR/R0, where ΔR and R0 represent the resistance change and the original resistance, respectively) decreases significantly with increasing temperature from -30 to 60 °C [Figure 4E], demonstrating a clear monotonic resistance-temperature dependence and a typical negative resistance temperature coefficient characteristic[32]. Calculated TCR values are -43% and -2% °C-1 in the temperature ranges of -30~10 and 10-60 °C, respectively, surpassing most reported temperature sensors [Figure 4F and Supplementary Table 5][33-39]. This exceptional thermal responsiveness is attributed to two main factors. Firstly, due to the narrow bandgap semiconducting behavior of MXene nanosheets[5], thermal excitation during heating provides electrons with enough energy to transition to higher energy states, increasing the carrier concentration. As a result, MXene nanosheet’s conductivity increases with rising temperature, enhancing electron transport efficiency. Secondly, migration rate of H+ ions, which are ionized from PA, accelerates with temperature, enhancing the temperature-sensing ability of the PCM organohydrogel. Thus, the combination of electron transitions and increased ion migration contributes to the superior temperature detection abilities of PCM organohydrogels. Moreover, stable and repeatable temperature-sensing behavior was confirmed in five cyclic tests, where the temperature was varied from 25 to -10, -20, and -30 °C [Figure 4G] and then from 25 to 40, 50 and 60 °C [Figure 4H], manifesting excellent reliability and electrical replicability of the PCM organohydrogel as a temperature sensor. Notably, the temperature sensor can also detect consecutive temperature variations, showcasing its practical applicability [Figure 4I]. For example, the temperature sensor successfully distinguished the consecutive thermal flows produced by a hair dryer set to low, medium, and high temperatures, illustrating the high sensitivity of the PCM organohydrogel temperature sensor due to the temperature-induced changes in electron transport efficiency and ion migration rates.
Owing to excellent conductivity and mechanical robustness, the PCM organohydrogel is highly suitable as a flexible strain sensor. Strain sensitivity is a crucial factor for evaluating the performance of strain sensors, which is typically quantified by calculating the gauge factor (GF) from the slope of ΔR/R0 as a function of the applied strain. As depicted in Figure 5A, the ΔR/R0 value increases with increasing the tensile strain and can be split into three distinct linear sensing regimes: a low GF of 0.91 in the strain range of 0%-1,000%, a medium GF of 1.67 in the range of 1,000%-2,300%, and an impressive GF of 3.24 in the range of 2,300%-2,800%, demonstrating high sensitivity and wide strain sensing window. Notably, the PCM organohydrogel exhibits an improved GF compared to the PC organohydrogel, indicating that the incorporation of MXene significantly enhances the strain sensitivity of the sensor. Encouragingly, compared with the organohydrogel without CS, the addition of CS in the organohydrogel system greatly improves the strain response behavior of the flexible strain sensor [Supplementary Figure 18]. The strain-resistance responsiveness of the PCM organohydrogel during the stretching process can be explained as follows: firstly, a large number of evenly dispersed MXene nanosheets interconnect with each other in the PCM organohydrogel matrix to form a complete conductive network framework for electron transport between MXene nanosheets, while the porous microstructure facilitates the effective transmission of free ions, endowing the PCM organohydrogel with favorable conductivity [Supplementary Figure 19A]. At small strains, the spacing between adjacent MXene nanosheets increases due to their slippage, and the reduction in the cross-sectional area of the hydrogel due to the Poisson effect decreases the transmission of free ions, leading to an increase in resistance [Supplementary Figure 19B]. As the strain further increases, the MXene nanosheets gradually separate from each other and the free ion conduction becomes slower, generating the discontinuous conductive pathways in the PCM organohydrogel and a higher GF [Supplementary Figure 19C]. Figure 5B shows that the electrical signals of the PCM organohydrogel remain nearly identical when stretched circularly at a 100% strain under applied stretching rates ranging from 50 to 400 mm·min-1, demonstrating the typical rate-independent strain response feature, which is important for the rapid and accurate detection of complex human movements[10]. The response and recovery time of the PCM organohydrogel are both less than 300 ms [Figure 5C], indicating excellent electromechanical performance. Stable and invertible output response signals can be recognized, regardless of whether the dynamic tensile testing is conducted under the small detection limit of 0.5% [Figure 5D], medium strain (100%-300%) [Figure 5E], and high strain (500%-900%) [Figure 5F], manifesting the reliable strain sensing ability and wide workable range of the PCM organohydrogel sensor. Moreover, output electrical signals remain stable during 2,000 consecutive stretching-releasing cycles at 50% strain, indicating excellent durability and reliability of the PCM organohydrogel [Figure 5G]. Notably, the healed PCM organohydrogel can generate stable and reproducible sensing signals during repeated stretching at 50% strain [Supplementary Figure 20], highlighting excellent self-healing ability and reliable strain-sensing performance. When compared to other reported hydrogel-based sensors, the PCM organohydrogel stands out due to its comprehensive properties, including strain range, maximum strain sensitivity, adhesion strength, self-HE, temperature range, and maximum TCR value [Figure 5H and Supplementary Table 6][40-47], making the PCM organohydrogel particularly well-suited for complex application scenarios.
Figure 5. Strain sensing performances of the PCM organohydrogel. (A) Sensitivity values of the PC and PCM organohydrogels; (B) ΔR/R0 of the PCM organohydrogel under continuous stretching-releasing cycles at the strain of 100% with different tensile rates; (C) Measurement of response/recovery time of the PCM organohydrogel under 1% strain at a tensile rate of 500 mm·min-1; Real-time sensing response of the PCM organohydrogel under (D) small strains, (E) medium strains, and (F) large strains; (G) Long-term stability of the PCM organohydrogel strain sensor over 2,000 stretching-releasing cycles at the strain of 50%; (H) Comparison of the PCM organohydrogel strain sensor with other reported hydrogel-based sensors in terms of strain, maximum strain sensitivity, adhesion strength, self-HE, temperature range, and maximum TCR value. PCM: PAM/CS/MXene; PC: PAM/CS; HE: healing efficiency; TCR: temperature coefficient of resistance.
Given its robust mechanical properties and excellent strain sensing performance, the PCM organohydrogel can be effectively assembled into wearable strain sensors for detecting complex physiological movements. Notably, outstanding adhesion characteristic of PCM organohydrogels allows it to directly adhere to human skin and conform to joint movements. As depicted in Figure 6A, subtle throat muscle activity, such as speaking, can be accurately and promptly recognized on account of the high sensitivity and rapid sensing capability of the PCM organohydrogel. Stable and reproducible response waveforms were observed when the volunteer repeatedly spoke the phrase “”, further demonstrating its excellent sensing stability and reliability. Thanks to its favorable environmental stability, the PCM organohydrogel was successfully adhered to the manipulator’s finger to precisely monitor bending deformation even in harsh environments at -30 °C [Figure 6B] and 60 °C [Figure 6C], which is extremely indispensable in practical applications. Additionally, the PCM organohydrogel maintained stable and consistent sensing response signals during knee bending deformation detection after healing [Figure 6D], underscoring the self-healing ability of the material and its ability to prolong sensor lifespan without compromising strain sensing performance. Furthermore, the PCM organohydrogel strain sensor can also be employed for information encryption via Morse code, enabling specific signal transmission through the arrangement of “dots” and “dashes”
Figure 6. Demonstration of PCM organohydrogel wearable strain sensors for monitoring human activities. (A) Repetitive sensing signals during speaking. ΔR/R0 of the PCM organohydrogel in response to finger bending at (B) low temperature and (C) high temperature environments; (D) Knee bending signal detection using the initial and healed PCM organohydrogel sensors; (E and F) Applications of the PCM organohydrogel in Morse code transmission. EMG signals recording from the (G) bicep and (H) forearm using the PCM organohydrogel; (I) Interfacial impedance comparison of the PCM organohydrogel electrodes and commercial gel electrodes; (J) EMG signals and SNR measured by PCM organohydrogel electrodes and commercial hydrogel electrodes; (K) Characteristic EMG signals corresponding to specific gestures; (L) EMG signals from the calf muscle area during jumping; Informed signed consent was obtained from the volunteer for the human biosignal detection experiments. PCM: PAM/CS/MXene; EMG: electromyography; SNR: signal-to-noise ratio.
Thanks to repeatable adhesion and favorable conductivity, the PCM organohydrogel can be effectively utilized as skin bioelectrodes for collecting high-quality electrophysiological signals. Circular epidermal electrodes were fabricated and applied to the skin of the bicep [Figure 6G], forearm [Figure 6H], and calf muscle regions of a volunteer to capture electromyography (EMG) signals generated by corresponding muscle contraction movements. Low interfacial impedance is crucial for ensuring high-quality sensing signals[48]. As shown in Figure 6I, interfacial impedance of the organohydrogel electrode in the physiologically relevant frequency range of 10-104 Hz is consistently lower than that of commercial gel electrodes. Remarkably, interfacial impedance of the organohydrogel electrode was found to be as low as
Ball game recognition has become increasingly important in the field of sports training. Five distinct ball sports (basketball shooting, volleyball bumping, backhand table tennis, badminton smashing, and bowling) can be efficiently recognized based on the seven-channel electrical sensing signals captured from seven different parts of the volunteer’s body (i.e., thumb, index finger, middle finger, ring finger, little finger, wrist, and elbow). Each set of response signals shows subtle variations for every repeated instance of the same ball sport [Supplementary Figures 24-28]. Impressively, standardized electrical response signals for each sport exhibit distinct differences [Figure 7A], highlighting significant potential for precise ball game recognition.
Figure 7. Design of a deep learning-assisted ball sports recognition system. (A) Standardized seven-channel response signals for five ball sports; (B) Schematic diagram showing the detection of signals from fingers, wrists, and elbows and the DLA framework using random forest; (C) Confusion matrix for recognizing the five ball sports actions; (D) t-SNE visualization of the training data for five different ball games; (E) Feature importance graph illustrating the key features for classification; (F) Learning curve showing the model’s performance over time. DLA: Deep learning algorithm; t-SNE: t-distributed stochastic neighborhood embedding.
Traditional recognition methods rely on manual extraction of shallow characteristics from raw sensor data, such as curvature of the back of the hand, perpendicularity between wrist and elbow, presenting certain challenges in achieving multi-target and high-precision recognition. By integrating ball game recognition with advanced DLAs, high-accuracy classification and recognition of ball games can be achieved, which holds immense potential for aiding athletes in evaluating and recognizing movement posture and diagnosing personal healthcare conditions. In an active monitoring mode, seven independent organohydrogel sensors were adhered to different parts of the volunteer’s body, including thumb, index finger, middle finger, ring finger, little finger, wrist, and elbow, forming a body-area sensor network [Figure 7B].
The initial dataset was split into 80% for model training and 20% for testing. The random forest algorithm was chosen to perform the classification tasks, leading to precise identification of various ball sports and evaluation of the model’s performance. The resulting confusion matrix shows a high overall recognition accuracy (100%), demonstrating the exceptional precision of the deep learning-assisted ball sports recognition system [Figure 7C]. Furthermore, the preprocessed data were input into t-distributed stochastic neighborhood embedding (t-SNE) for dimensionality reduction and clustering. As shown in Figure 7D, the captured data for different ball actions are clearly distinguished and the data points corresponding to the same ball action colored identically, categorizing the data into five distinct groups. Figure 7E presents the feature importance analysis, quantifying the contribution of each input signal to the model’s predictions. This highlights the significant role of signal 3 in the classification process, providing valuable insights into the underlying principles of the model’s decision-making. Finally, the learning curve in Figure 7F illustrates the model’s performance across both the training and validation datasets as the number of training samples increases. The progressive convergence of the curve emphasizes the model’s outstanding generalization ability, effectively avoiding overfitting or underfitting.
CONCLUSIONS
In summary, MXene-nanocomposited organohydrogels with ultrastretchability, robust adhesiveness, self-healing ability, and environmental stability were successfully fabricated to realize dual sensory functionalities. By incorporating CS-encapsulated MXene nanosheets into a PAM-crosslinked network, the system’s conductivity was significantly enhanced. The CS molecular forms a protective shell around the MXene nanosheets via hydrogen bonds and electrostatic interactions, improving their stability and dispersibility within the organohydrogel. Thanks to abundance of reversible noncovalent bonds, the PCM organohydrogel exhibits ultrastretchability (2,800%), reversible adhesiveness, and self-healing ability, making it ideal for constructing conformal electrode interfaces for high-efficiency electrophysiological signal detection. The synergistic hydrogen bonds between GL, PA, and water molecules endow the PCM organohydrogel with environmental adaptability (-30~60 °C), ensuring stable performance across a wide range of operating conditions and long-term applicability. Notably, the multimodal organohydrogel demonstrates reliable temperature response ability with a satisfactory thermosensation (-43% °C-1) and excellent strain sensing performances with high sensitivity of 3.24 at strains of 2,300%-2,800% and high stability. Impressively, the PCM sensor can detect and differentiate a broad range of human activities and subtle electrophysiological signals. Moreover, the organohydrogel sensing system assisted by DLAs achieves 100% accuracy in ball sports recognition, highlighting its promising potential in intelligent wearable electronics.
DECLARATIONS
Acknowledgements
The authors would like to thank the National Supercomputing Centre in Zhengzhou and the funding of Zhengzhou University for the DFT calculations.
Authors’ contributions
Formal analysis, investigation, data curation, writing - original draft, and visualization: Huang, M.
Formal analysis, software: Liu, S.
Investigation, formal analysis, and validation: Chi, Y.
Visualization, validation, formal analysis: Li, J.
Conceptualization, methodology, resources, writing - review and editing, and supervision: Sun, H.
Methodology, and writing - review and editing: Dong, L.
Conceptualization, methodology, resources, writing - review and editing, supervision, and funding acquisition: Liu, H.
Methodology, writing - review and editing, and funding acquisition: Liu, C.
Funding acquisition: Shen, C.
Availability of data and materials
All data are available in the main text or the Supplementary Materials. Information requests should be directed to the corresponding authors upon reasonable request.
Financial support and sponsorship
This research was financially supported by the National Natural Science Foundation of China (52203108, 52373093), Excellent Youth Found of Natural Science Foundation of Henan Province (242300421062), Central Plains Youth Top notch Talent Program, the Key Scientific Research Projects of Colleges and Universities in Henan Province, China (25A430011), and the 111 project (D18023).
Conflicts of interest
All authors declared that there are no conflicts of interest.
Ethical approval and consent to participate
All human experiments involving strain sensor testing were conducted with informed written consent from the participant (an author of this study). The study complied with local regulations and ethical standards, involving only non-invasive monitoring of bodily motion and physical signals. No sensitive personal data or commercial interests were involved, and the procedure caused no harm to participants, thus qualifying for ethical approval exemption.
Consent for publication
Not applicable.
Copyright
© The Author(s) 2025.
Supplementary Materials
REFERENCES
1. Lin, F.; Yang, W.; Lu, B.; et al. Muscle-inspired robust anisotropic cellulose conductive hydrogel for multidirectional strain sensors and implantable bioelectronics. Adv. Funct. Mater. 2025, 35, 2416419.
2. Li, H.; Cao, J.; Wan, R.; et al. PEDOTs-based conductive hydrogels: design, fabrications, and applications. Adv. Mater. 2025, 37, e2415151.
3. Zhang, Y.; Tan, Y.; Lao, J.; Gao, H.; Yu, J. Hydrogels for flexible electronics. ACS. Nano. 2023, 17, 9681-93.
4. Jin, J.; Wang, S.; Zhang, Z.; Mei, D.; Wang, Y. Progress on flexible tactile sensors in robotic applications on objects properties recognition, manipulation and human-machine interactions. Soft. Sci. 2023, 3, 8.
5. Liu, Y.; Tian, G.; Du, Y.; et al. Highly stretchable, low-hysteresis, and adhesive TA@MXene-composited organohydrogels for durable wearable sensors. Adv. Funct. Mater. 2024, 34, 2315813.
6. Niu, Y.; Liu, H.; He, R.; et al. The new generation of soft and wearable electronics for health monitoring in varying environment: from normal to extreme conditions. Mater. Today. 2020, 41, 219-42.
7. Zhu, C.; Chen, G.; Li, S.; et al. Breathable ultrathin film sensors based on nanomesh reinforced anti-dehydrating organohydrogels for motion monitoring. Adv. Funct. Mater. 2024, 34, 2411725.
8. Chen, G.; Zhang, Y.; Li, S.; et al. Flexible artificial tactility with excellent robustness and temperature tolerance based on organohydrogel sensor array for robot motion detection and object shape recognition. Adv. Mater. 2024, 36, e2408193.
9. Huang, X.; Zheng, Z.; Wang, H.; et al. A freeze-resistant, highly stretchable and biocompatible organohydrogel for non-delayed wearable sensing at ultralow-temperatures. Adv. Funct. Mater. 2024, 34, 2312149.
10. Sun, H.; Han, Y.; Huang, M.; et al. Highly stretchable, environmentally stable, self-healing and adhesive conductive nanocomposite organohydrogel for efficient multimodal sensing. Chem. Eng. J. 2024, 480, 148305.
11. Wu, J.; Huang, W.; Wu, Z.; et al. Hydrophobic and stable graphene-modified organohydrogel based sensitive, stretchable, and self-healable strain sensors for human-motion detection in various scenarios. ACS. Mater. Lett. 2022, 4, 1616-29.
12. Wang, W.; Zhou, H.; Xu, Z.; Li, Z.; Zhang, L.; Wan, P. Flexible conformally bioadhesive MXene hydrogel electronics for machine learning-facilitated human-interactive sensing. Adv. Mater. 2024, 36, e2401035.
13. Chae, A.; Murali, G.; Lee, S.; et al. Highly oxidation-resistant and self-healable MXene-based hydrogels for wearable strain sensor. Adv. Funct. Mater. 2023, 33, 2213382.
14. Gong, T.; Li, Z. N.; Liang, H.; et al. High-sensitivity wearable sensor based on a MXene nanochannel self-adhesive hydrogel. ACS. Appl. Mater. Interfaces. 2023, 15, 19349-61.
15. Zhang, H.; Shen, H.; Lan, J.; Wu, H.; Wang, L.; Zhou, J. Dual-network polyacrylamide/carboxymethyl chitosan-grafted-polyaniline conductive hydrogels for wearable strain sensors. Carbohydr. Polym. 2022, 295, 119848.
16. Ji, D.; Park, J. M.; Oh, M. S.; et al. Superstrong, superstiff, and conductive alginate hydrogels. Nat. Commun. 2022, 13, 3019.
17. Nie, Y.; Yue, D.; Xiao, W.; et al. Anti-freezing and self-healing nanocomposite hydrogels based on poly(vinyl alcohol) for highly sensitive and durable flexible sensors. Chem. Eng. J. 2022, 436, 135243.
18. Zhang, Q.; Liu, X.; Zhang, J.; Duan, L.; Gao, G. A highly conductive hydrogel driven by phytic acid towards a wearable sensor with freezing and dehydration resistance. J. Mater. Chem. A. 2021, 9, 22615-25.
19. Hu, R.; Zhao, J.; Wang, Y.; Li, Z.; Zheng, J. A highly stretchable, self-healing, recyclable and interfacial adhesion gel: preparation, characterization and applications. Chem. Eng. J. 2019, 360, 334-41.
20. Chen, Z.; Liu, S.; Kang, P.; et al. Decoupled temperature–pressure sensing system for deep learning assisted human–machine interaction. Adv. Funct. Mater. 2024, 34, 2411688.
21. Yang, C.; Huang, W.; Lin, Y.; et al. Stretchable MXene/carbon nanotube bilayer strain sensors with tunable sensitivity and working ranges. ACS. Appl. Mater. Interfaces. 2024, 16, 30274-83.
22. Dai, N.; Lei, I. M.; Li, Z.; Li, Y.; Fang, P.; Zhong, J. Recent advances in wearable electromechanical sensors - moving towards machine learning-assisted wearable sensing systems. Nano. Energy. 2023, 105, 108041.
23. Wang, M.; Li, L.; Zhang, T. Hysteresis-free, fatigue-resistant and self-adhesive conductive hydrogel electronics towards multimodal wearable application. Nano. Energy. 2024, 126, 109586.
24. Li, Q.; Zhi, X.; Xia, Y.; et al. Ultrastretchable high-conductivity MXene-based organohydrogels for human health monitoring and machine-learning-assisted recognition. ACS. Appl. Mater. Interfaces. 2023, 15, 19435-46.
25. Pi, M.; Qin, S.; Wen, S.; et al. Rapid gelation of tough and anti-swelling hydrogels under mild conditions for underwater communication. Adv. Funct. Mater. 2023, 33, 2210188.
26. Huang, Y.; Xiao, L.; Zhou, J.; et al. Strong tough polyampholyte hydrogels via the synergistic effect of ionic and metal–ligand bonds. Adv. Funct. Mater. 2021, 31, 2103917.
27. Han, Y.; Wang, Z.; Sun, H.; et al. Temperature-tolerant versatile conductive zwitterionic nanocomposite organohydrogel toward multisensory applications. ACS. Appl. Mater. Interfaces. 2024, 16, 38606-19.
28. Qin, Z.; Sun, X.; Yu, Q.; et al. Carbon nanotubes/hydrophobically associated hydrogels as ultrastretchable, highly sensitive, stable strain, and pressure sensors. ACS. Appl. Mater. Interfaces. 2020, 12, 4944-53.
29. Zhao, H.; Hao, S.; Fu, Q.; et al. Ultrafast fabrication of lignin-encapsulated silica nanoparticles reinforced conductive hydrogels with high elasticity and self-adhesion for strain sensors. Chem. Mater. 2022, 34, 5258-72.
30. Fan, X.; Ke, T.; Gu, H. Multifunctional, ultra-tough organohydrogel E-skin reinforced by hierarchical goatskin fibers skeleton for energy harvesting and self-powered monitoring. Adv. Funct. Mater. 2023, 33, 2304015.
31. Ni, Y.; Zang, X.; Yang, Y.; et al. Environmental stability stretchable organic hydrogel humidity sensor for respiratory monitoring with ultrahigh sensitivity. Adv. Funct. Mater. 2024, 34, 2402853.
32. Zhang, X.; Cui, C.; Chen, S.; et al. Adhesive ionohydrogels based on ionic liquid/water binary solvents with freezing tolerance for flexible ionotronic devices. Chem. Mater. 2022, 34, 1065-77.
33. Hao, S.; Meng, L.; Fu, Q.; Xu, F.; Yang, J. Low-temperature tolerance and conformal adhesion zwitterionic hydrogels as electronic skin for strain and temperature responsiveness. Chem. Eng. J. 2022, 431, 133782.
34. Gu, J.; Huang, J.; Chen, G.; et al. Multifunctional poly(vinyl alcohol) nanocomposite organohydrogel for flexible strain and temperature sensor. ACS. Appl. Mater. Interfaces. 2020, 12, 40815-27.
35. Zhang, Z.; Tang, L.; Chen, C.; et al. Liquid metal-created macroporous composite hydrogels with self-healing ability and multiple sensations as artificial flexible sensors. J. Mater. Chem. A. 2021, 9, 875-83.
36. Chen, H.; Huang, J.; Liu, J.; et al. High toughness multifunctional organic hydrogels for flexible strain and temperature sensor. J. Mater. Chem. A. 2021, 9, 23243-55.
37. Xie, Z.; Li, H.; Mi, H.; Feng, P.; Liu, Y.; Jing, X. Freezing-tolerant, widely detectable and ultra-sensitive composite organohydrogel for multiple sensing applications. J. Mater. Chem. C. 2021, 9, 10127-37.
38. Zhou, L.; Li, Y.; Xiao, J.; et al. Liquid metal-doped conductive hydrogel for construction of multifunctional sensors. Anal. Chem. 2023, 95, 3811-20.
39. Liu, Z.; Wang, Y.; Ren, Y.; et al. Poly(ionic liquid) hydrogel-based anti-freezing ionic skin for a soft robotic gripper. Mater. Horiz. 2020, 7, 919-27.
40. Chen, L.; Chang, X.; Wang, H.; Chen, J.; Zhu, Y. Stretchable and transparent multimodal electronic-skin sensors in detecting strain, temperature, and humidity. Nano. Energy. 2022, 96, 107077.
41. Zhang, B.; Zhang, X.; Song, H.; Nguyen, D. H.; Zhang, C.; Liu, T. Strong-weak response network-enabled ionic conductive hydrogels with high stretchability, self-healability, and self-adhesion for ionic sensors. ACS. Appl. Mater. Interfaces. 2022, 14, 32551-60.
42. Hao, S.; Dai, R.; Fu, Q.; et al. A robust and adhesive hydrogel enables interfacial coupling for continuous temperature monitoring. Adv. Funct. Mater. 2023, 33, 2302840.
43. Ni, Y.; Zang, X.; Chen, J.; et al. Flexible MXene-based hydrogel enables wearable human–computer interaction for intelligent underwater communication and sensing rescue. Adv. Funct. Mater. 2023, 33, 2301127.
44. Lei, T.; Wang, Y.; Feng, Y.; et al. PNIPAAm-based temperature responsive ionic conductive hydrogels for flexible strain and temperature sensing. J. Colloid. Interface. Sci. 2025, 678, 726-41.
45. Qu, X.; Sun, H.; Kan, X.; et al. Temperature-sensitive and solvent-resistance hydrogel sensor for ambulatory signal acquisition in “moist/hot environment”. Nano. Res. 2023, 16, 10348-57.
46. Qu, X.; Liu, J.; Wang, S.; et al. Photothermal regulated multi-perceptive poly(ionic liquids) hydrogel sensor for bioelectronics. Chem. Eng. J. 2023, 453, 139785.
47. Li, Y.; Yang, D.; Wu, Z.; et al. Self-adhesive, self-healing, biocompatible and conductive polyacrylamide nanocomposite hydrogels for reliable strain and pressure sensors. Nano. Energy. 2023, 109, 108324.
Cite This Article

How to Cite
Download Citation
Export Citation File:
Type of Import
Tips on Downloading Citation
Citation Manager File Format
Type of Import
Direct Import: When the Direct Import option is selected (the default state), a dialogue box will give you the option to Save or Open the downloaded citation data. Choosing Open will either launch your citation manager or give you a choice of applications with which to use the metadata. The Save option saves the file locally for later use.
Indirect Import: When the Indirect Import option is selected, the metadata is displayed and may be copied and pasted as needed.
About This Article
Special Issue
Copyright
Data & Comments
Data
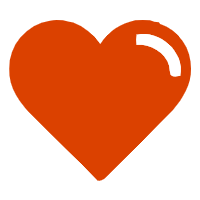
Comments
Comments must be written in English. Spam, offensive content, impersonation, and private information will not be permitted. If any comment is reported and identified as inappropriate content by OAE staff, the comment will be removed without notice. If you have any queries or need any help, please contact us at [email protected].